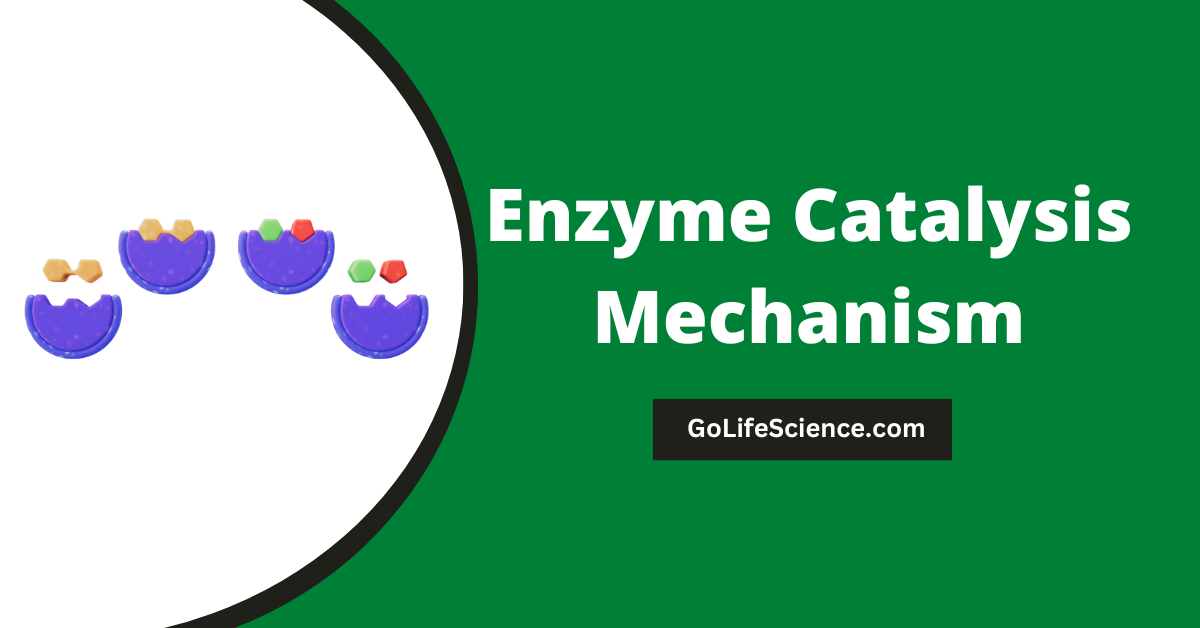
Enzymes play a crucial role in the biological world, orchestrating countless chemical reactions that sustain life. These remarkable proteins act as catalysts, accelerating reactions that would otherwise occur too slowly to support life processes. Understanding the enzyme catalysis mechanism is crucial for advancements in medicine, biotechnology, and various industrial applications. This article delves into the intricate world of enzyme catalysis, exploring its mechanisms, types, and significance in life sciences.
Table of Contents
What Are Enzymes?
Enzymes are specialized proteins that facilitate biochemical reactions without being consumed in the process. They are highly specific, each designed to catalyze a particular reaction or set of reactions. This specificity is due to the unique three-dimensional structure of the enzyme, which includes an active site where the substrate (the molecule upon which the enzyme acts) binds.
Key Characteristics of Enzymes
- Catalytic Efficiency: Enzymes can increase reaction rates by several orders of magnitude.
- Specificity: Each enzyme typically catalyzes a specific reaction or a set of closely related reactions.
- Regulation: Enzyme activity can be regulated by various factors, including pH, temperature, and the presence of inhibitors or activators.
The Enzyme Catalysis Mechanism
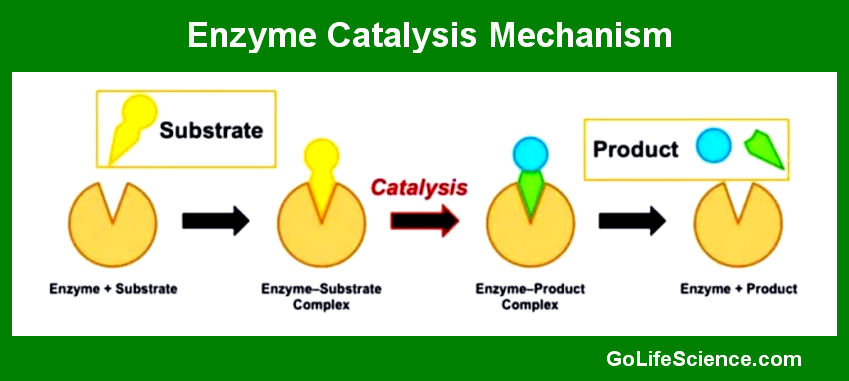
The enzyme catalysis mechanism can be understood through several key concepts: the active site, substrate binding, transition state stabilization, and product release.
- The Active Site: The active site is a region on the enzyme where the substrate binds and the catalytic reaction occurs. It is typically a small pocket or cleft on the enzyme’s surface, formed by a unique arrangement of amino acids. The active site’s structure is complementary to the substrate, ensuring specificity.
- Substrate Binding: Substrate binding involves the formation of an enzyme-substrate (ES) complex. This binding is facilitated by non-covalent interactions such as hydrogen bonds, ionic bonds, and van der Waals forces. The induced fit model suggests that the enzyme’s active site undergoes conformational changes upon substrate binding, optimizing the fit and enhancing catalytic efficiency.
- Transition State Stabilization: The transition state is a high-energy, unstable state that reactants must pass through to form products. Enzymes stabilize this transition state, lowering the activation energy required for the reaction to proceed. This stabilization is achieved through precise positioning of the substrate and catalytic residues within the active site, as well as the formation of transient covalent bonds.
- Product Release: Once the reaction is complete, the products are released from the active site, and the enzyme returns to its original state, ready to catalyze another reaction. The release of products is often facilitated by conformational changes in the enzyme.
Types of Enzyme Catalysis
Enzymes employ various catalytic mechanisms to facilitate reactions. These mechanisms can be broadly categorized into several types:
- Acid-Base Catalysis: In acid-base catalysis, the enzyme donates or accepts protons (H⁺) to stabilize the transition state. This can involve specific amino acid residues in the active site, such as aspartate, glutamate, histidine, or lysine, which can act as proton donors or acceptors.
- Covalent Catalysis: Covalent catalysis involves the formation of a transient covalent bond between the enzyme and the substrate. This bond stabilizes the transition state and facilitates the reaction. A common example is the use of nucleophilic residues like serine or cysteine to form covalent intermediates.
- Metal Ion Catalysis: Many enzymes require metal ions (e.g., Zn²⁺, Mg²⁺, Fe²⁺) for catalytic activity. These metal ions can stabilize negative charges, participate in redox reactions, or act as Lewis acids to polarize substrates.
- Electrostatic Catalysis: Electrostatic catalysis involves the stabilization of charged intermediates or transition states through electrostatic interactions. This can include the stabilization of negatively charged intermediates by positively charged residues in the active site.
- Proximity and Orientation Effects: Enzymes bring substrates into close proximity and orient them in a specific manner, increasing the likelihood of a successful reaction. This reduces the entropy of the system and enhances the reaction rate.
Factors Affecting Enzyme Catalysis
Several factors influence the rate and efficiency of enzyme-catalyzed reactions:
- Temperature: Enzyme activity generally increases with temperature, up to a point known as the optimum temperature. Beyond this point, the enzyme may denature, losing its catalytic activity.
- pH: Each enzyme has an optimal pH at which it functions most efficiently. Deviations from this pH can lead to changes in the enzyme’s structure and a decrease in activity.
- Substrate Concentration: The rate of an enzyme-catalyzed reaction increases with substrate concentration, up to the point where the enzyme becomes saturated. At this point, the reaction rate reaches its maximum (Vmax).
- Enzyme Concentration: Increasing the concentration of the enzyme generally increases the reaction rate, provided there is an excess of substrate.
- Inhibitors and Activators: Inhibitors are molecules that decrease enzyme activity, while activators enhance it. Inhibitors can be competitive (binding to the active site) or non-competitive (binding elsewhere on the enzyme).
Enzyme Kinetics
Enzyme kinetics is the study of the rates of enzyme-catalyzed reactions. The Michaelis-Menten equation is a fundamental model describing the relationship between substrate concentration and reaction rate:
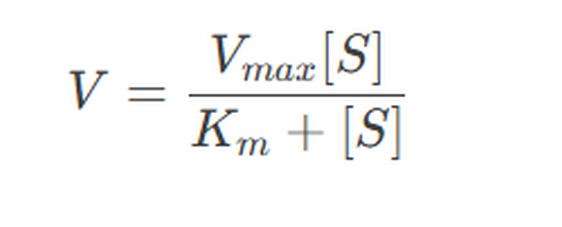
Where:
- VV is the reaction rate.
- VmaxVmax is the maximum reaction rate.
- [S][S] is the substrate concentration.
- KmKm is the Michaelis constant, representing the substrate concentration at which the reaction rate is half of VmaxVmax.
Lineweaver-Burk Plot
The Lineweaver-Burk plot is a double reciprocal plot used to linearize the Michaelis-Menten equation:
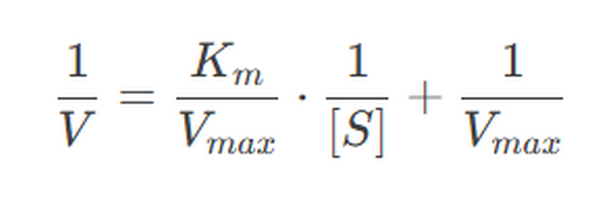
This plot is useful for determining Vmax and Km from experimental data.
Enzyme Regulation
Enzyme activity is tightly regulated to maintain metabolic homeostasis. Regulation can occur at various levels:
- Allosteric Regulation: Allosteric regulation involves the binding of a molecule (allosteric effector) at a site other than the active site, causing conformational changes that alter enzyme activity. Allosteric enzymes often have multiple subunits and exhibit cooperative binding.
- Covalent Modification: Covalent modification involves the addition or removal of chemical groups (e.g., phosphorylation, acetylation) to the enzyme, altering its activity. This is often reversible and regulated by other enzymes.
- Zymogen Activation: Some enzymes are synthesized as inactive precursors (zymogens) that require proteolytic cleavage to become active. This mechanism prevents premature enzyme activity and allows for precise control.
- Feedback Inhibition: In feedback inhibition, the end product of a metabolic pathway inhibits an enzyme early in the pathway, preventing overproduction of the product.
Applications of Enzyme Catalysis
Understanding enzyme catalysis has numerous practical applications:
- https://golifescience.com/oxidative-phosphorylation/Medicine: Enzymes are targets for many drugs. Inhibitors can be designed to block the activity of specific enzymes involved in disease processes, such as protease inhibitors used in HIV treatment.
- Biotechnology: Enzymes are used in various biotechnological processes, including the production of biofuels, pharmaceuticals, and food products. For example, amylases are used in the production of high-fructose corn syrup.
- Industrial Chemistry: Enzymes are employed in industrial processes to catalyze reactions under mild conditions, reducing energy consumption and waste production. Examples include the use of lipases in detergent formulations.
- Environmental Applications: Enzymes are used in bioremediation to break down pollutants and in waste treatment to degrade organic matter.
Conclusion
Enzyme catalysis is a cornerstone of biochemistry, enabling the complex web of reactions that sustain life. By understanding the mechanisms of enzyme catalysis, scientists can harness these powerful biological catalysts for a wide range of applications, from medicine to industrial chemistry. As research continues to uncover the intricacies of enzyme function, the potential for new and innovative uses of enzymes in science and industry is boundless.
References
- Berg, J. M., Tymoczko, J. L., & Stryer, L. (2015). Biochemistry (8th ed.). W.H. Freeman and Company.
- Nelson, D. L., & Cox, M. M. (2017). Lehninger Principles of Biochemistry (7th ed.). W.H. Freeman and Company.
- Voet, D., & Voet, J. G. (2011). Biochemistry (4th ed.). Wiley.
- Fersht, A. (1999). Structure and Mechanism in Protein Science: A Guide to Enzyme Catalysis and Protein Folding. W.H. Freeman and Company.