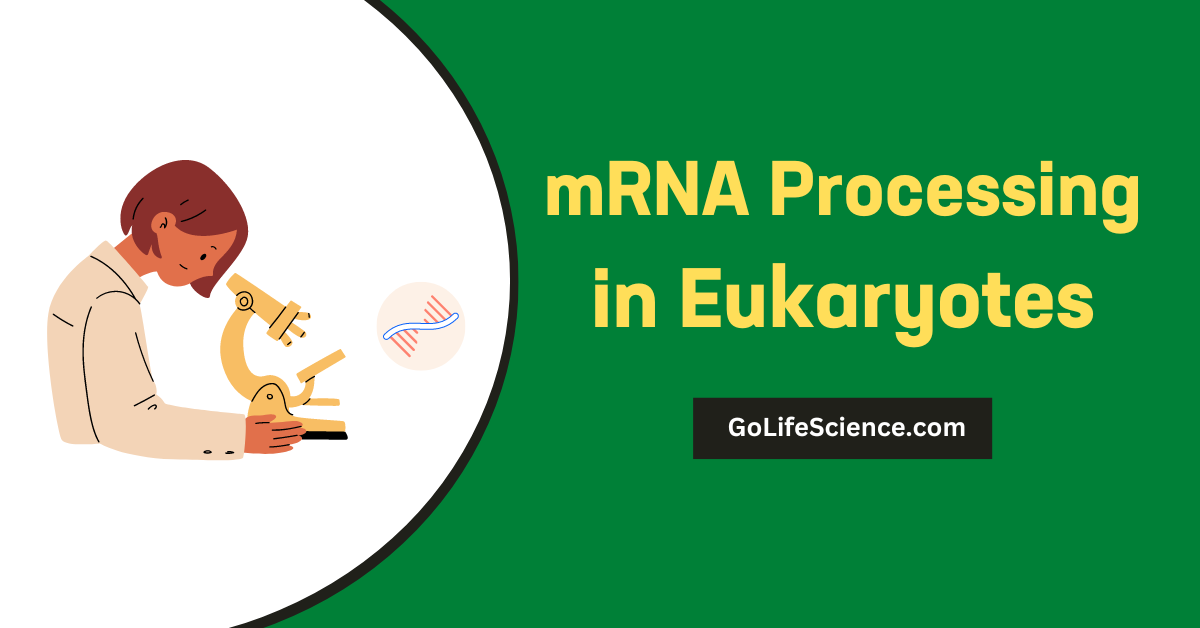
In the intricate world of molecular biology, the process of gene expression stands as a fundamental pillar of life. Messenger RNA (mRNA) is at the heart of this process. It serves as a crucial intermediary. DNA stores the genetic code. Functional proteins carry out cellular activities. In eukaryotic cells, the journey from gene to protein is complex. It involves a series of sophisticated modifications collectively known as mRNA processing.
This article delves deep into the fascinating realm of mRNA processing in eukaryotes. It explores the essential steps and regulatory mechanisms. The significance of this process in maintaining cellular function and overall health is also discussed. The nucleus transcribes a gene and then exports mature mRNA. We will unravel the complex choreography of molecular events. These events ensure the fidelity and efficiency of gene expression.
As we navigate through the intricacies of 5′ capping, splicing, and polyadenylation, we will also examine how these processes function. We will explore how disruptions in these processes can lead to various diseases. This highlights the critical importance of mRNA processing in human health. Finally, we’ll look at cutting-edge research in this field. This offers a glimpse into the future of molecular biology. It also explores potential applications in medicine and biotechnology.
Whether you’re a student, researcher, or simply curious about the molecular basis of life, this comprehensive guide to mRNA processing in eukaryotes promises to enlighten and inspire. It reveals the elegant complexity of one of nature’s most fundamental processes.
Table of Contents
Overview of mRNA
Messenger RNA (mRNA) serves as the template for protein synthesis, carrying genetic information from DNA to the ribosome. In eukaryotes, the path from gene to functional mRNA involves several critical steps:
- Transcription: The process begins in the nucleus, where RNA polymerase II transcribes a gene into a precursor mRNA (pre-mRNA).
- Processing: The pre-mRNA undergoes three main modifications:
- 5′ capping
- Splicing
- 3′ polyadenylation
- Export: The mature mRNA is transported from the nucleus to the cytoplasm.
- Translation: Ribosomes read the mRNA sequence to synthesize proteins.
A mature eukaryotic mRNA typically has the following structure:
- 5′ cap: A modified guanine nucleotide at the 5′ end
- 5′ untranslated region (UTR): Regulatory sequences before the start codon
- Coding sequence: The portion that codes for the protein
- 3′ UTR: Regulatory sequences after the stop codon
- Poly-A tail: A string of adenine nucleotides at the 3′ end
Here’s a simplified diagram of a mature mRNA structure:
5′ cap – 5′ UTR – START CODON – CODING SEQUENCE – STOP CODON – 3′ UTR – Poly-A tail 3′
To understand how gene expression is controlled, it is important to know how mRNA is structured. This knowledge also aids in making RNA-based therapies.
Pre-mRNA Processing Steps
1. 5′ Capping
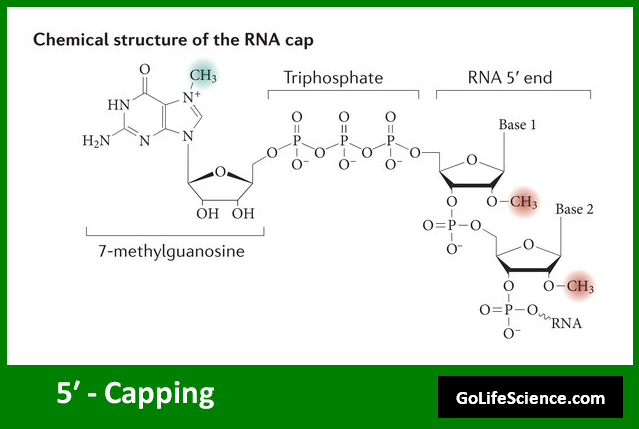
The 5′ capping process is the first modification applied to the newly transcribed pre-mRNA. A 7-methylguanosine (m7G) cap is added to the 5′ end of the RNA molecule as part of this process. The capping process occurs co-transcriptionally. It begins shortly after the start of transcription. This happens when the RNA chain is about 20-30 nucleotides long.
The 5′ capping process involves three main enzymatic steps:
- Triphosphatase activity: Removes the terminal phosphate from the 5′ end of the pre-mRNA.
- Guanylyltransferase activity: Adds a guanosine monophosphate (GMP) to the diphosphate end.
- Methyltransferase activity: Methylates the guanine at the N7 position.
The resulting structure is often referred to as a “cap-0” structure. In some cases, additional methylations can occur on the first nucleotide of the RNA. This leads to a “cap-1” structure. Further methylation on the second nucleotide results in a “cap-2” structure.
Functions of the 5′ cap:
- Protection from exonucleases: The cap prevents degradation of the mRNA by 5′ to 3′ exonucleases.
- Splicing enhancement: It promotes the efficiency of splicing, particularly of the first intron.
- Nuclear export: Export factors recognize the cap, facilitating the transport of mature mRNA to the cytoplasm.
- Translation initiation: Eukaryotic initiation factors (eIFs) recognize the cap in the cytoplasm, which promotes ribosome recruitment and translation initiation.
The importance of the 5′ cap is underscored by its conservation across eukaryotes. It plays a crucial role in multiple steps of mRNA metabolism.
2. Splicing
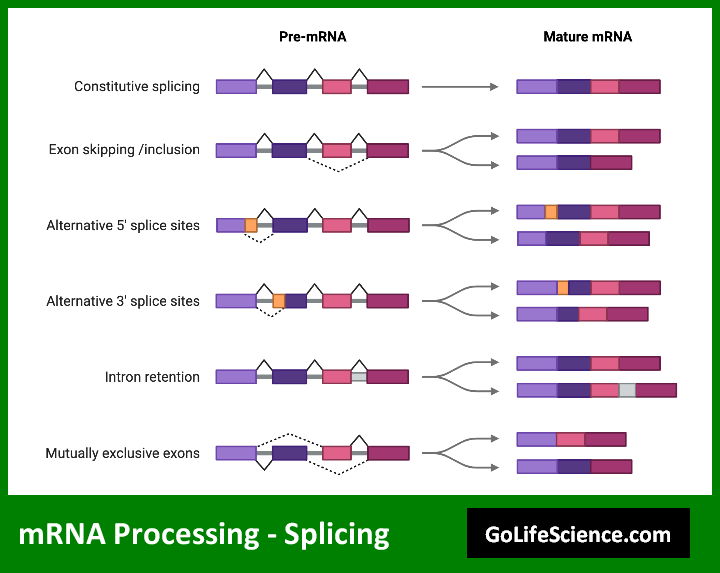
Splicing is an important part of processing mRNA; it gets rid of non-coding sequences (introns) and joins coding sequences (exons). This process is carried out by a large ribonucleoprotein complex called the spliceosome.
The splicing process occurs in several steps:
- Recognition of splice sites: The spliceosome identifies specific sequences at the intron-exon boundaries.
- Spliceosome assembly: Various small nuclear ribonucleoproteins (snRNPs) and other proteins assemble on the pre-mRNA.
- Two transesterification reactions:
- First reaction: The 5′ splice site cleaves, forming a lariat structure at the 5′ end of the intron.
- The second reaction involves cleaving the 3′ splice site and joining the exons.
- The process involves the release of the spliced mRNA and the disassembly of the spliceosome.
The splicing reaction can be represented by the following chemical equation:
Pre-mRNA + ATP → Mature mRNA + Intron lariat + ADP + Pi
Types of splicing:
- Constitutive splicing: The final mRNA includes all exons and removes all introns.
- Alternative splicing: Different combinations of exons can be included or excluded, leading to multiple mRNA isoforms from a single gene.
A limited number of genes can produce a greatly increased protein diversity through alternative splicing. In humans, it’s estimated that over 95% of multi-exon genes undergo alternative splicing.
Regulation of splicing:
Splicing is regulated by various factors, including:
- Cis-acting elements: sequences within the pre-mRNA that can enhance or suppress splicing.
- Trans-acting factors: proteins that bind to the cis-acting elements and influence spliceosome assembly.
These factors interact to set the final splicing pattern. This lets genes be expressed in specific tissues and at certain stages of development.
3. 3′ Polyadenylation
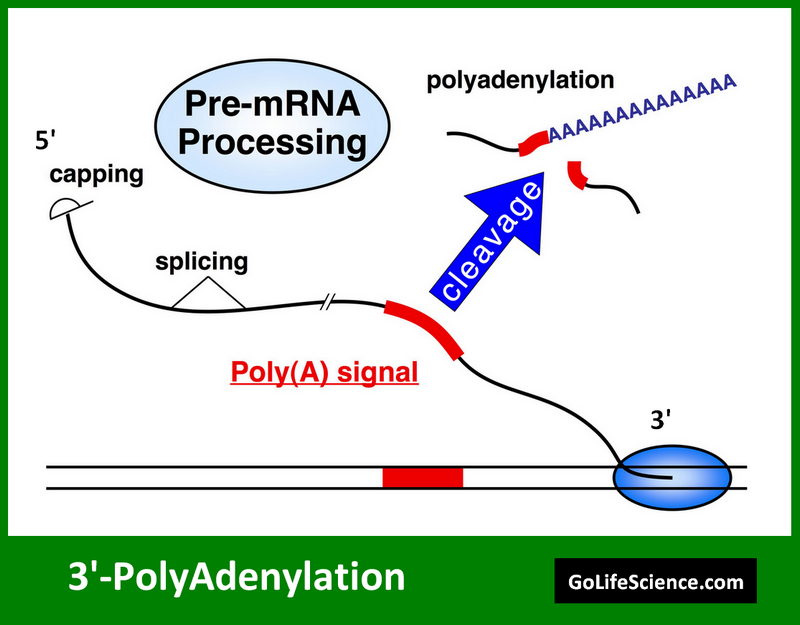
3′ polyadenylation is the last big step in pre-mRNA processing. This is where a poly(A) tail is added to the 3′ end of the mRNA. This process is crucial for mRNA stability, export, and translation efficiency.
The 3′ polyadenylation process involves several steps:
- The cleavage and polyadenylation specificity factor (CPSF) recognizes specific sequence elements in the 3′ UTR.
- Endonucleolytic cleavage: The pre-mRNA is cleaved at a specific site, typically 10-30 nucleotides downstream of the polyadenylation signal (usually AAUAAA).
- Synthesis of the poly(A) tail: The poly(A) polymerase (PAP) adds a string of adenine nucleotides. It attaches them to the cut 3′ end of the mRNA.
The poly(A) tail is typically 150-250 nucleotides long in mammals. However, its length can vary between species. It can even differ between different mRNAs within the same cell.
Functions of the poly(A) tail:
- mRNA stability: Protects the mRNA from 3′ to 5′ exonucleolytic degradation.
- Nuclear export: Facilitates the transport of mature mRNA from the nucleus to the cytoplasm.
- Translation efficiency: Interacts with proteins that enhance translation initiation.
- Controlling mRNA half-life: The poly(A) tail gets shorter in the cytoplasm. This shortening can signal the cell to break down the mRNA.
Regulation of polyadenylation:
There are several ways to control polyadenylation:
- Alternative polyadenylation: Many genes have multiple polyadenylation sites, leading to mRNAs with different 3′ UTR lengths.
- Tissue-specific factors: Some proteins can enhance or suppress polyadenylation in specific cell types.
- Developmental regulation: The efficiency of polyadenylation can change during different stages of development.
The following table summarizes the key features of the three main pre-mRNA processing steps:
Process | Location | Key Enzymes | Main Functions |
---|---|---|---|
5′ Capping | Nucleus (co-transcriptional) | Triphosphatase, Guanylyltransferase, and Methyltransferase | Protection, splicing enhancement, export, and translation initiation |
Splicing | Nucleus | Spliceosome complex | Intron removal, exon joining, and protein diversity |
3′ Polyadenylation | Nucleus | CPSF, Poly(A) polymerase | mRNA stability, export, translation efficiency |
Regulation of mRNA Processing
The regulation of mRNA processing is a complex and dynamic process that allows cells to fine-tune gene expression in response to various stimuli. This regulation occurs at multiple levels and involves numerous factors:
- Transcriptional Regulation
- Promoter strength: Affects the rate of pre-mRNA synthesis.
- Transcription factors: Can influence the recruitment of processing machinery.
- Splicing Regulation
- Splice site strength: Affects the efficiency of spliceosome assembly.
- Splicing enhancers and silencers: Cis-acting elements that promote or inhibit splicing.
- SR proteins and hnRNPs: Trans-acting factors that bind to regulatory elements.
- Tissue-specific splicing factors: Allow for cell-type-specific alternative splicing patterns.
- Polyadenylation Regulation
- Poly(A) signal variants: Different signal sequences can affect cleavage and polyadenylation efficiency.
- RNA-binding proteins: Can modulate the recognition of poly(A) signals.
- Alternative polyadenylation: Selection of different poly(A) sites can produce mRNAs with different 3′ UTR lengths.
- RNA Editing
- Adenosine-to-Inosine (A-to-I) editing: Can alter splicing patterns or coding sequences.
- Cytidine-to-Uridine (C-to-U) editing: Can change the meaning of codons or create stop codons.
- Epigenetic Regulation
- Histone modifications: Can affect the accessibility of DNA to transcription and processing machinery.
- DNA methylation: Can influence splicing patterns by modulating the rate of RNA polymerase II elongation.
- Cellular Stress Responses
- Heat shock: Can lead to widespread changes in splicing patterns.
- Oxidative stress: Can affect the activity of splicing factors.
- Feedback Loops
- Autoregulation: Some splicing factors can regulate their own expression through alternative splicing.
The interplay between these regulatory mechanisms allows for precise control of gene expression. For instance, changing the 3′ UTR length through alternative polyadenylation can alter the stability and localization of mRNA. Additionally, adding or removing certain exons through alternative splicing can significantly influence how proteins work.
Understanding these regulatory mechanisms is crucial to comprehend how cells respond to their environment. Dysregulation of these mechanisms can lead to disease states. It also makes therapeutic interventions possible, like splice-switching oligonucleotides that can change the way splicing patterns work in genetic disorders.
Importance in Gene Expression
mRNA processing plays a pivotal role in gene expression, serving as a critical link between transcription and translation. Its importance can be understood through several key aspects:
- Diversification of the Proteome
- Alternative splicing allows a single gene to produce multiple protein isoforms.
- This greatly expands the functional diversity of the proteome without increasing genome size.
- Example: The Drosophila Down syndrome cell adhesion molecule (Dscam) gene can potentially generate over 38,000 different protein isoforms through alternative splicing.
- Regulation of Gene Expression
- mRNA processing provides additional layers of control over gene expression.
- Different mRNA isoforms can have varying stability, localization, or translation efficiency.
- This allows for fine-tuning of protein levels in response to cellular needs.
- Cellular Differentiation and Development
- Tissue-specific and developmental stage-specific splicing patterns are crucial for proper organism development.
- Changes in splicing patterns can drive cell differentiation processes.
- Response to Environmental Stimuli
- External signals can rapidly modulate mRNA processing.
- This allows cells to quickly adapt their protein repertoire to changing conditions.
- Quality Control
- Splicing, in particular, serves as a quality control checkpoint in mRNA processing.
- Improperly processed mRNAs can be degraded, preventing the production of potentially harmful proteins.
- Evolutionary Adaptability
- Changes in mRNA processing patterns can lead to phenotypic differences between species.
- This provides a mechanism for evolutionary adaptation without necessarily requiring changes to coding sequences.
- Energy Efficiency
- By removing introns, which can make up a large portion of pre-mRNAs, cells save energy in mRNA export and translation processes.
To illustrate the quantitative impact of mRNA processing on gene expression, consider the following data:
Process | Impact on Gene Expression |
---|---|
Alternative Splicing | 95% of human multi-exon genes |
Alternative Polyadenylation | ~ 70% of human genes |
RNA Editing | Thousands of human genes |
These numbers underscore the pervasive nature of mRNA processing in shaping the human transcriptome and proteome.
The importance of mRNA processing in gene expression is further emphasized by the severe consequences that can arise from its dysregulation, which we’ll explore in the next section on diseases associated with mRNA processing defects.
Diseases Associated with mRNA Processing Defects
Defects in mRNA processing can lead to a wide range of diseases, highlighting the critical importance of these mechanisms in maintaining cellular function and overall health. Here are some key categories of diseases associated with mRNA processing defects:
- Neurodegenerative Disorders
- Spinal Muscular Atrophy (SMA): Caused by mutations in the SMN1 gene, affecting splicing of multiple genes.
- Amyotrophic Lateral Sclerosis (ALS): Some forms are associated with mutations in RNA processing genes like TDP-43 and FUS.
- Frontotemporal Dementia (FTD): Often involves defects in RNA processing, including mutations in the MAPT gene affecting tau protein splicing.
- Cancer
- Chronic Lymphocytic Leukemia: Often involves mutations in splicing factor SF3B1.
- Myelodysplastic Syndromes: are frequently associated with mutations in splicing factors like SF3B1, SRSF2, and U2AF1.
- Various Solid Tumors: Aberrant splicing of genes like BRCA1, FGFR2, and CD44 has been implicated in different cancers.
- Genetic Disorders
- Duchenne Muscular Dystrophy: This often involves mutations that affect splicing of the dystrophin gene.
- Cystic Fibrosis: Some mutations in the CFTR gene affect splicing, leading to non-functional protein.
- Beta-Thalassemia: This can be caused by mutations that create aberrant splice sites in the beta-globin gene.
- Autoimmune Diseases
- Systemic Lupus Erythematosus: Associated with defects in RNA processing and increased expression of Alu elements.
- Multiple Sclerosis: Some risk variants affect splicing of the IL7R gene.
- Metabolic Disorders
- Familial Hypercholesterolemia: Some cases involve mutations affecting splicing of the LDL receptor gene.
- Congenital Disorders of Glycosylation: Can involve defects in splicing of genes involved in glycosylation pathways.
- Developmental Disorders
- Prader-Willi Syndrome: Involves loss of expression of paternally expressed genes, some of which are involved in RNA processing.
- Rett Syndrome: While primarily caused by mutations in MECP2, some cases involve aberrant splicing.
The following table summarizes some key diseases and their associated mRNA processing defects:
Disease | Type of mRNA Processing Defect | Affected Gene(s) |
---|---|---|
Spinal Muscular Atrophy | Splicing | SMN1 |
Chronic Lymphocytic Leukemia | Splicing | SF3B1 |
Duchenne Muscular Dystrophy | Splicing | DMD (Dystrophin) |
Cystic Fibrosis | Splicing | CFTR |
Beta-Thalassemia | Splicing | HBB (Beta-globin) |
Familial Hypercholesterolemia | Splicing | LDLR |
These diseases illustrate the wide-ranging impacts of mRNA processing defects on human health. The diversity of affected tissues and systems underscores the fundamental importance of proper mRNA processing in cellular function.
Understanding the molecular basis of these diseases has opened up new avenues for therapeutic interventions. For example:
- Antisense oligonucleotides: These can be used to modulate splicing patterns, as in the case of nusinersen for spinal muscular atrophy.
- Small molecule splicing modulators: Compounds that can correct aberrant splicing are being developed for various diseases.
- Gene therapy: Approaches to correct defective genes or provide functional copies are being explored.
Research into mRNA processing defects not only enhances our understanding of disease mechanisms but also provides insights into normal cellular function and gene regulation. This knowledge is crucial for developing new diagnostic tools and targeted therapies for a wide range of disorders.
Recent Advances in mRNA Processing Research
The field of mRNA processing has seen significant advancements in recent years, driven by technological innovations and increased understanding of its importance in cellular function. Here are some key areas of recent progress:
- Single-cell RNA Sequencing
- Allows for the analysis of mRNA processing events at the individual cell level.
- Has revealed unexpected heterogeneity in splicing patterns within seemingly homogeneous cell populations.
- Example: A 2021 study used single-cell RNA-seq to map splicing QTLs in human immune cells, revealing cell-type-specific effects of genetic variants on splicing.
- Long-read Sequencing Technologies
- Enables the sequencing of full-length transcripts, providing a more complete picture of splicing patterns and isoform diversity.
- Has led to the discovery of novel splice isoforms and gene fusion events.
- A 2020 study using long-read sequencing in human brain tissue identified over 13,000 novel splice junctions and 3,000 novel genes.
- CRISPR-Cas9 Screening
- Allows for high-throughput functional analysis of splicing regulators and cis-regulatory elements.
- Has identified new factors involved in splicing regulation and disease mechanisms.
- In 2019, a CRISPR-Cas9 screen identified hundreds of genes that modulate splicing of the NGLY1 gene, mutations in which cause a rare genetic disorder.
- Structural Biology of the Spliceosome
- Cryo-electron microscopy has provided high-resolution structures of the spliceosome at different stages of the splicing reaction.
- These structures have revealed the intricate mechanisms of splice site recognition and catalysis.
- A landmark 2018 study provided the first near-atomic resolution structure of the entire yeast spliceosome.
- RNA Modifications in mRNA Processing
- Growing evidence for the role of RNA modifications (the “epitranscriptome”) in regulating splicing and other processing events.
- m6A modification has been shown to influence splice site choice and mRNA stability.
- A 2020 study found that m6A modification regulates alternative polyadenylation by recruiting YTHDC1 to sites of 3′ end processing.
- Liquid-Liquid Phase Separation in mRNA Processing
- Recognition that many RNA processing events occur within membraneless organelles formed by phase separation.
- This concept has provided new insights into the organization and regulation of mRNA processing.
- A 2019 study showed that phase separation of the splicing factor hnRNPA2 regulates the splicing of the FGFR2 gene.
- Therapeutic Applications
- Development of antisense oligonucleotides and small molecules to modulate splicing in various diseases.
- Advances in mRNA vaccines, which rely on optimized mRNA processing for efficacy.
- The success of nusinersen for spinal muscular atrophy has spurred research into similar approaches for other splicing-related diseases.
Here’s a table summarizing some key recent findings:
Year | Discovery | Potential Impact |
---|---|---|
2021 | Single-cell mapping of splicing QTLs | Personalized medicine approaches for splicing-related diseases |
2020 | Long-read sequencing reveals thousands of novel splice junctions in human brain | Improved understanding of neurological disorders |
2019 | CRISPR screen identifies regulators of NGLY1 splicing | New therapeutic targets for NGLY1 deficiency |
2018 | Near-atomic resolution structure of yeast spliceosome | Rational design of splicing modulators |
2020 | m6A regulates alternative polyadenylation | New layer of gene expression control |
2019 | Phase separation regulates FGFR2 splicing | Novel mechanism of splicing regulation |
These advances are not only enhancing our understanding of fundamental biology but also opening up new possibilities for diagnostic and therapeutic interventions. As research continues, we can expect further insights into the complexities of mRNA processing and its role in health and disease.
Final words about mRNA processing
The field of mRNA processing in eukaryotes represents a fascinating intersection of molecular biology, genetics, and cellular physiology. From the initial steps of 5′ capping to the intricate dance of splicing and the final addition of the poly(A) tail, each stage of mRNA processing plays a crucial role in gene expression and cellular function.
Key takeaways from our exploration include:
- mRNA processing is a multi-step process involving 5′ capping, splicing, and 3′ polyadenylation, each with specific functions in mRNA stability, export, and translation.
- Alternative splicing and polyadenylation greatly increase the diversity of the proteome, allowing for complex regulation of gene expression.
- Regulation of mRNA processing occurs at multiple levels, involving both cis-acting elements and trans-acting factors, allowing for fine-tuned control of gene expression.
- Defects in mRNA processing are associated with a wide range of human diseases, from neurodegenerative disorders to cancer, highlighting its critical importance in maintaining cellular health.
- Recent technological advances, including single-cell RNA sequencing, long-read sequencing, and high-resolution structural biology, are providing unprecedented insights into the mechanisms and regulation of mRNA processing.
- These advances are opening up new avenues for therapeutic interventions, such as splice-switching oligonucleotides and small molecule modulators of mRNA processing.
As we look to the future, the field of mRNA processing continues to hold great promise. The ongoing integration of high-throughput technologies, computational biology, and mechanistic studies is likely to reveal even more complexities in how cells regulate gene expression through mRNA processing. Moreover, the growing understanding of how mRNA processing goes awry in disease states is paving the way for novel therapeutic strategies.
The study of mRNA processing serves as a powerful reminder of the intricate mechanisms that underlie life at the molecular level. As we continue to unravel these complexities, we not only gain a deeper appreciation for the elegance of cellular processes but also equip ourselves with the knowledge to address some of the most challenging issues in human health and disease.
Frequently Asked Questions (FAQs)
What is mRNA processing in eukaryotes?
mRNA processing in eukaryotes involves modifying the primary mRNA transcript to form mature mRNA, which includes capping, splicing, and adding a poly-A tail.
How does alternative splicing contribute to protein diversity?
Alternative splicing allows a single gene to produce multiple mRNA isoforms by selectively including or excluding certain exons. This process can lead to the production of different protein variants from the same gene, each potentially having distinct functions or regulatory properties. For example, the human Dscam gene can potentially generate over 38,000 protein isoforms through alternative splicing.
What is the difference between pre-mRNA and mature mRNA?
Pre-mRNA is the initial transcript produced by RNA polymerase II during transcription. It contains both introns (non-coding regions) and exons (coding regions). Mature mRNA is the final product after processing, which includes 5′ capping, splicing (removal of introns), and 3′ polyadenylation. Mature mRNA is ready for export to the cytoplasm and translation into protein.
What is the purpose of 5′ capping?
The 5′ cap protects the mRNA from degradation, assists in ribosome binding for translation, and aids in the export of mRNA from the nucleus.
What happens during RNA splicing?
During RNA splicing, introns (non-coding regions) are removed from the primary mRNA transcript, and exons (coding regions) are joined together to form a continuous coding sequence.
What is the poly-A tail and its function?
The poly-A tail is a string of adenine nucleotides added to the 3′ end of the mRNA. It stabilizes the mRNA molecule, aids in its export from the nucleus, and enhances translation efficiency.
Why is mRNA processing important?
mRNA processing is crucial for producing a mature, stable, and translatable mRNA. It ensures that only the correct coding sequences are translated into proteins, which is vital for proper gene expression and cellular function.